Photinus pyralis (Common Eastern Firefly)
Luciferase
Suzanna Bator '20 and Myanna Cook '21
Contents:
I. Introduction
Model View:
(Common Eastern) Firefly luciferase is a 62 kDa oxidoreductase that catalyzes the production of light. With a two step chemical process, luciferase is used to emit light in fireflies to attract mates. As an enzyme, luciferase uses both MgATP and oxygen to oxidize the enzyme�s substrate luciferin. This oxidation leads to the emission of the yellow-green glow most kids chase after in summer nights. Although this enzyme has rather slow kinetics, luciferase is able to produce one photon of light for each molecule of luciferin that is oxidized. An in depth mechanism for luciferase activity is shown in Figure 1.
Luciferase is frequently used as a reporter gene in studies since the emission of light can be measured easily; the more light emitted (measured in relative luminometer units, RLU), the more the gene is expressed. The enzyme can also be used to quantify ATP utilization. Beyond luciferase�s assay capabilities, the enzyme has a lot of sequence homology with fatty-acyl coenzyme A (CoA) ligases and peptide synthetases as these enzymes activate their substrates by catalyzing an enzyme-bound adenylate intermediate. These enzymes, like luciferase, catalyze enzyme-bound adenylated intermediates by linking the carboxyl of its substrate to the phosphoryl moiety of AMP, and transfers this activated substrate to an acceptor like CoA.
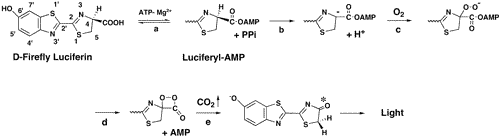
Figure 1. Luciferase chemical reaction. In the
first (activation) step, luciferin (Enzyme +Luc-COOH) is activated
through the acylation of the carboxylate group with the ?-phosphate of
ATP, making the enzyme-bound luciferyl adenylate (Enzyme:Luc-CO-AMP)
and releasing a pyrophosphate. The second (oxidation) step oxides the
luciferyl adenylate with molecular oxygen and releases CO2
This oxidation excites an electron creating an excited state product
(Enzyme: Luc=O*) that then decays to the ground state (Luc=O) and
emits light (hv). Image taken from Branchini et al. 2003.
II. General Structure
Model View:
P.
pyralis Luciferase (as crystallized by Conti et. al., 1996) is
comprised of
: a 432-residue N-terminus domain
and a 108-residue C-terminus domain.
The
domain includes three subdomains: a distorted, anti-parallel
β-barrel (Subdomain
) and two β-sheets bordered by α-helices called β-sheet
A and β-sheet B (Subdomains
and
). Subdomains A and B create a long surface groove that
Subdomain C closes.
The
domain is separated from the main N-terminus
domain by a wide cleft. The C-terminus
domain is often compared to a lid on top of the β-barrel. Two
short, antiparallel β-strands and a three-stranded mixed β-sheet with
three α helices packed at the sides create the α+β structure of this
domain.
are marked here, consistent with the coloring above.
III. Conserved Residues
Model View:
Firefly
luciferases have a lot of primary sequence and mechanistic
similarities to peptide synthetases and acyl-CoA ligases. Both of
these enzymes activate their substrates into enzyme-bound adenylate
intermediates just like luciferase. Within a representative set of 38
enzymes (consisting of 9 firefly luciferases, 18 acyl-CoA ligases, and
the activated domain from 11 peptide synthetases; enzymes chosen by
Conti et al., 1996) from multiple organisms that catalyze the
acylation reaction (using 15 unique substrates), there are extensive
regions of primary sequence similarity. Out of the total 550 residues
in this firefly luciferase, 75 residues are identical in at least 50%
of the 38 enzyme set; however, there are only
-- G200(not located in
experiment), K206, E344,
D422, R437(not
located in experiment), G446 and E455 -- which most likely play a key role in
ATP binding and adenylate formation. There are other conserved
sequences motifs with invariant residues, one of which is a motif of
198[STG]-[STG]-G-[ST]-[TSE]-[GS]-x(L)-[PALIVM]-K206 (blue residues are specific to this luciferase;
brackets are alternative residues and x is a hypervariable position;
residues 199-203 were not located in
this experiment). G200 and K206
are invariant, and mutagenesis of the
to an R was shown to dramatically decrease enzyme activity.
Other alterations did not have as drastic of an effect. There are two
other motifs found in this enzyme set: a
340[YFW]-[GASW]-x(L)-[TSA]-E344
and a
420[STA]-[GRK]-D422. Residues E344
and D422 are invariant.
IV. Active Site
Model View:
The proposed
by Conti et al. 1996 includes 3 of the 7 invariant residues mentioned above (the
3 invariant residues are labelled red).
The other 6 active site residues are identical in at least 50% of the
38 enzymes. In other ATP and GTP-binding proteins there is a classical
mononucleotide-binding (Rossmann) fold. The motif for this fold is
G-x-x-x-x-G-K-[STG], and unfortunately is disordered in this
luciferase structure; however, there is interpretable electron density
in the N-terminus
,
and
residues.
faces the cleft between the two domains and is readily
exposed to the solvent (8% w/v PEG 8000, 10% glycerol, 12.5% ethylene
glycol and 100 mM Tris-HCl pH 7.8). S198
is
to a carboxylate oxygen of E344. D422
is exposed to the solvent and its carboxylate groups
to the side chain of Y340, a well conserved residue. The hydroxyl group of S420
has the potential to
with the backbone nitrogens of both D422 and G421.
The cleft is
too large for the substrates and interactions with the residues to
occur. Since the invariant residues lay on inner surfaces of the two
domains within the realm of the cleft and the cleft is too large, it
is thought that the domains substantially change their conformation to
sandwich the substrates after binding. A tight pocket must be formed
by the two domains because light production needs water excluded from
the active site to prevent intermolecular quenching of the
excited-state product.
Further
research by Branchini et al. has shown a
within the N-terminus domain consisting of 15 residues. Of
these 15 residues, 6 ( K529, H245,
T343, G315,
G316, G341)
are found in at least 50% of the 38 enzyme set from the study by
Conti. et al.
is thought to orient the carboxylate of D-LH2 in
proximity to the α-phosphate of the ATP.
is conserved in all luciferases and this glycine is located
in a
that most likely allows for conformational flexibility that
is necessary for enzyme activity and substrate binding. Branchini et.
al. suggest that
makes electrostatic interactions with both D-LH2
and ATP (K529 side chain not fully available). The side chain of R218 is
to the hydroxyl of S347 and the main carbonyl group
of A348. This interaction is thought to bring α helix 7 of Subdomain A into
with the β-hairpin motif (residues 340-344; mentioned in Conserved Residues section).
V. Color Emission Determination
Model View:
Differences in
the structure of particular luciferase enzymes play a key role in the
color of the emitted light. Branchini et al., 2017 have found a
single amino acid residue that responsible for the difference in color
emission from yellow-green to orange:
The change of
to an F. The researchers used site-directed mutagenesis to
show the 13nm redshift that F255 causes. This position 255
residue is located proximal to the substrate/emitter binding site.
Another change from the positively charged residue to a negatively charged D residue
also resulted in red bioluminescence.
From this finding, Branchini et al. speculate that R218 maintains polarity and rigidity in the emitter binding site.
This polarity and rigidity is necessary for the yellow-green emission in firefly luciferase.
Changes in
polarity of the active site regulate changes in emission colors,
specifically changing the H-bond H2O networks. Branchini
et. al. found that the lower the pH, the more redshift light emission
occurred; changes in the H2O network may be responsible for
this effect. In redshifted mutants, the H2O network loses
one H-bond that then allows inhibitor DLSA, a dehydroluciferin-AMP
analog(an intermediate in the luciferase reaction), to interact with
. This is thought to be the possible difference between the
H-bond networks of the red and green light emitting luciferases. Minor
disruptions in the H-bond pattern like the one mentioned above could
allow excited state proton transfer and quenching green light
emission.
VI. References
Branchini, B.R., Magyar, R.A., Murtiashaw, M.H. and Portier, N.C. (2001).
The role of active site residue arginine 218 in firefly luciferase bioluminescence. Biochem. 40, 2410-2418.
Branchini, B.R., Southworth, T.L., Murtiashaw, M.H., Boije, H. and Fleet, S.E. (2003). A
mutagenesis study of the putative luciferin binding site residues of firefly luciferase.
Biochem. 42, 10429-10436.
Branchini, B.R., Southworth, T.L., Fontaine, D.M., Murtiashaw, M.H., McGurk, A., Talukder,
M.H., Qureshi, R., Yetil, D., Sundlov, J.A. and Gulick, A.M. (2017). Cloning of the orange light-producing luciferase from Photinus scintillans--a new proposal on how bioluminescence color is determined.
Photochem.
Photobio. 93, 479-485.
Conti, E., Franks, N.P. and Brick, P.
(1996). Crystal structure of firefly luciferase throws light on
a superfamily of adenylate-forming enzymes. Structure.
4, 287-298.
Nakatsu, T., Ichiyama, S., Hiratake, J.,
Saldanha, A., Kobashi, N., Skakta, K. and Kato, H. (2006).
Structural basis for the spectral difference in luciferase
bioluminescence. Nature. 440, 372-376.
Schmelz, S. and Naismith, J.H. (2009).
Adenylate-forming enzymes. Struct. Bio. 19, 666-671.
Back to Top