Polyethylene terephthalate
degrading hyrdrolyase enzyme from Ideonella Sakaiensis
Meheret Ourgessa '23 and Beimnet Kassaye '23
Contents:
I. Introduction
Polyethylene terephthalate (PET) is the most abundant type of plastic
in the world. It is used to make single-use bottles, packaging,
containers, and clothing. PET degrades at a very slow rate under
normal conditions, and this property has made it a major pollutant to
both land and water. Chemical recycling is the primary way of
degrading and depolymerizing PET, but the process is not
cost-effective since raw PET is cheaper than its recycled counterpart.
Analysis of PET contaminated matter from a recycling plant led to the
discovery of a bacterial species that uses PET as a carbon source. The
bacterium, Ideonella sakaiensis, secrets the enzyme
polyethylene terephthalate hydrolase (PETase) to hydrolyze the ester
bond in PET and convert it to BHET (Bis(2-hydroxyethyl) terephthalate)
and MHET (Mono-(2-hydroxyethyl)terephthalic acid). The bacteria also
produces MHETase, an enzyme that further breaks down MHET into the
monomers terephthalic acid and ethylene glycol.
While PETase from Ideonella sakaiensis has common
structural and functional properties with other hydrolases (such as
cutinases and lipases), it also has special features that allow it to
act on PET at room temperature. Research on the mechanism of the
enzymatic action is still under study, but it has already led to the
discovery of mutants which degrade PET with higher efficiency.

Figure 1. Scheme of polyethylene terephthalate degradation by PETase
into BHET(Bis(2-hydroxyethyl) terephthalate) and
MHET(Mono-(2-hydroxyethyl)terephthalic acid). MHETase further breaks
down MHET into the monomers TPA(terephthalic acid) and EG(ethylene
glycol).
II. General Structure
A member of the α/β-hydrolase family, PETase has a core containing
eight
and six
in its tertiary structure. The protein adopts an
α/β-hydrolase fold as would be predicted from its sequence homology
with proteins from the cutinase and lipase families. The surface of PETase is
polarized to highly acidic and basic areas with an isoionic point of
9.6. The weight of the protein is 31.46 kDa with a residue count of
265.
Within the active-site, the catalytic triad conserved across
cutinases and lipases is also present in PETase. In this protein,
comprise the catalytic triad. These catalytic residues
reside on loops. The nucleophilic serine residue occupies a highly
conserved position called the nucleophilic/catalytic elbow. This
structure has the consensus sequence (G-X1-S-X2-G). While X1 in
cutinases and lipases is usually occupied with phenylalanine or
histidine, in PETase, the position is occupied by tryptophan
giving the enzyme an extended hydrophobic surface next to
the active site.
Compared to its closest cutinases homologs, PETase has a broader
active-site cleft, and, at its broadest point, approaches three times
the width of the corresponding structure in the Thermobifida fusca
cutinase, another enzyme known for its ability to hydrolyze PET. This broadening happened with minimal rearrangement of
adjacent loops and the secondary structure; only a single amino acid
substitution from phenylalanine to serine (ser160) in the active site
seems to have caused it. The
of PETase is then formed between two tryptophan residues
(Trp159 and Trp185) and the novel Ser160.
PETase has two disulfide bonds, one that is adjacent to the active
site and another one close to the C terminus of the protein. The first
disulfide bond is between
and connects
, stabilizing the whole molecule. PETase's second disulfide
bond,
, is next to the active site and
a hidden site in β-sheet 7, which is found immediately below
the active site, to a loop that connects β-Sheet 8 with α-Helix 5.
This loop has the catalytic histidine His237. The disulfide bond
anchors the loop and stabilizes the histidine and the entire catalytic
triad. This stabilization allows for higher flexibility at room
temperature, which is a property absent in other thermophilic
hydrolases.
III. PET Binding and Degradation Mechanism
IFD (Induced-fit Docking) protocol has been used to predict
PET-PETase binding modes. It is thought that in a productive
PET-binding event, Ser160 is positioned at a distance of 5.1 Å from
the carbonyl carbon of PET. Within the same model, His237, positioned 3.9 Å from
Ser160, would
the serine residue for nucleophilic attack through a hydrogen
bond. Further, Asp206 provides
to His237 from a distance of 2.8 Å.
A
of PETase bound to a ligand, HMET (1-(2-hydroxyethyl)
4-methyl terephthalate), elucidates the exact mechanism of PET
degradation. HMET is a substrate analog here since, like PET, it has
an ester bond moiety and an aryl group. This structure
contains an
to inactivate the enzyme and determine the ligand-bound
structure. PET degradation seems to be achieved by the hydroxyl group
of the activated Ser160 attacking the carbonyl carbon of HEMT (and,
thus, likely PET too) in a nucleophilic acyl substitution mechanism.
During this attack, the ester group approaches the enzyme with the
carbonyl oxygen facing the
formed by Tyr87 and Met161
(Figure 2). These residues are both within
of the carbonyl carbon being attacked in HMET. The
positively charged nitrogen atoms in the oxyanion hole can stabilize
the partial negative charge on the carbonyl oxygen of the ester.
Leu208 and Met161 further provide
to HMET.
, a part of the active-site cleft, rotates as PET is bound in order to form aromatic
interactions with the molecule. The reorientation of Trp185 in the
ligand-bound model of PETase also suggests that the movement of this
residue opens up the active-site of the enzyme. Additionally,
seems to allow this rotation or wobbling of Trp185 and is therefore an
important feature of PETase.
After the first nucleophilic attack by the activated Ser160, a water
molecule will complete the cleavage of the ester bond (now between
Ser160 and the substrate) which leads to the formation of tereaphtalic acid.
The aromatic portion of the
terephthalic acid moiety then strengthens its hydrophobic interactions
(base stacking) with the indole ring in Trp185. This base stacking
will induce an angular change to the conformation of the substrate
initiating product release (Figure 2).
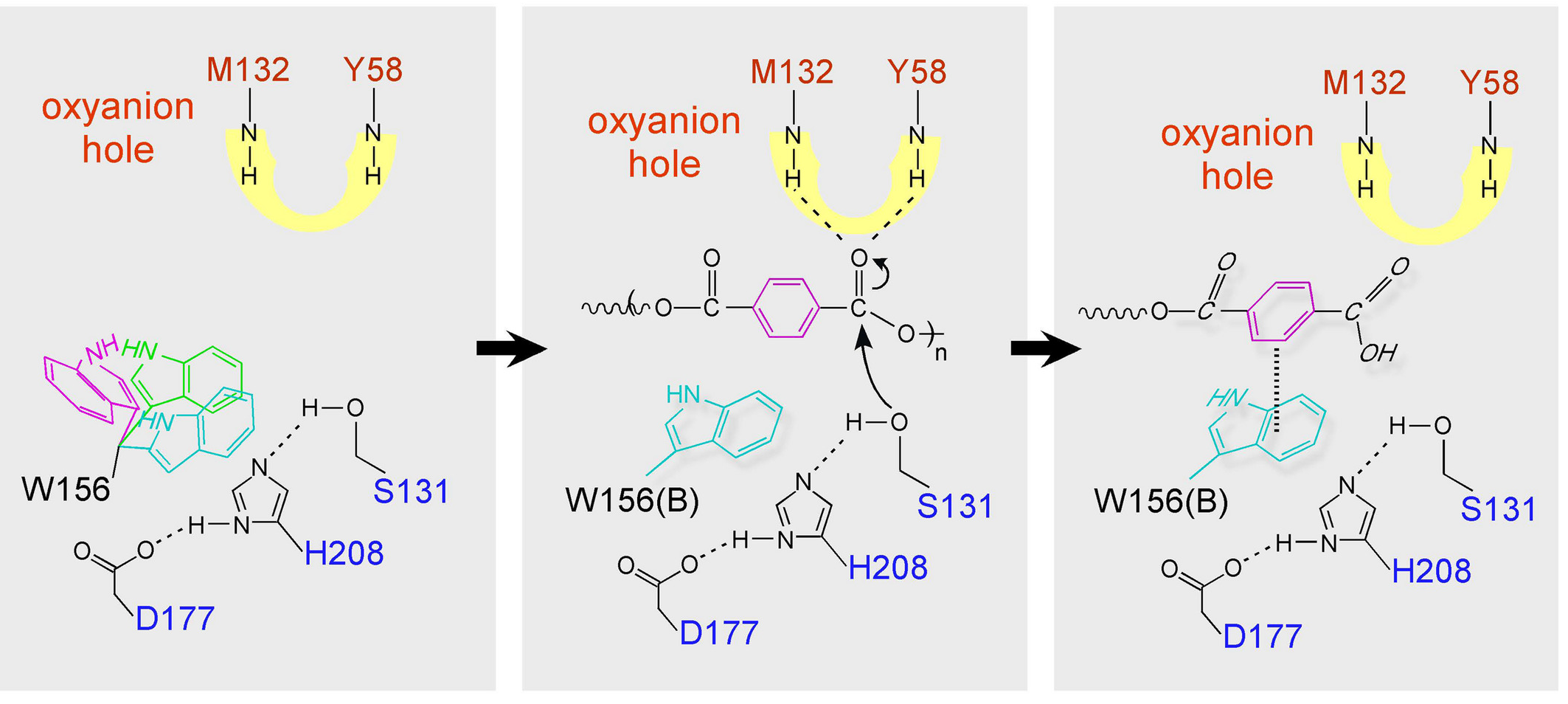
Figure 2. Binding and degradation of the analogous substrate HMET by
PETase. Interactions with the protein during product release are also
shown. All amino acid position numbers in this figure are shifted down
by 29 (for example, Y58 is the same as the residue Tyr87 presented in
the text).
IV. Improving Degradation
Specific mutations to the amino acids of PETase enhance degradation.
A double mutant with S238F/W159H outperforms the wild type at
degrading PET that has higher crystallinity like the one found in
plastic bottles. Like cutinases, the mutant had a narrower active site
that gave it better crystallinity reduction and product release. The
S238F mutation introduced a ring that allows for more hydrophobic
interactions with the surrounding terephthalate, and the W159H
substitution reduced steric hindrance and deepened the space in the
active site for PET binding.
Another substitution L117F was made at a position outside of the
active site channel. The change from the aliphatic to the
aromatic phenylalanine allowed for faster PET binding and release of
products. This mutant also outperformed the wild type PETase; its
degradation rate was 2.1 times that of the wild type. Mutation of
, found close to the active site, to the ringed phenylalanine gave
better PET binding, and the rate of degradation increased by 2.5
folds. The aromaticity of phenylalanine enhances degradation because
ring stacking between the amino acid residue and terephthalate
increases binding of the enzyme to PET.
The improved degradation by these protein-engineered mutants is
evidence that the activity of PETase could be enhanced and more PET
could be recycled faster.
V. References
Austin, H. P., Allen, M. D., Donohoe, B. S., Rorrer, N.
A., Kearns, F. L., Silveira, R. L., � Beckham, G. T. (2018).
Characterization and engineering of a plastic-degrading aromatic
polyesterase. Proceedings of the National Academy of Sciences,
115(19). https://doi.org/10.1073/pnas.1718804115
Chen, C. C., Han, X., Ko, T. P., Liu, W., & Guo,
R. T. (2018). Structural studies reveal the molecular mechanism of
PETase. The FEBS Journal , 285(20), 3717�3723.
https://doi.org/10.1111/febs.14612
Fecker, T., Galaz-Davison, P., Engelberger, F.,
Narui, Y., Sotomayor, M., Parra, L. P., & Ram�rez-Sarmiento, C.
A. (2018). Active Site Flexibility as a Hallmark for Efficient PET
Degradation by I. sakaiensis PETase. Biophysical Journal ,
114(6), 1302�1312. https://doi.org/10.1016/j.bpj.2018.02.005.
Ma, Y., Yao, M., Li, B., Ding, M., He, B., Chen, S.,
� Yuan, Y. (2018). Enhanced Poly(ethylene terephthalate) Hydrolase
Activity by Protein Engineering. Engineering, 4(6),
888�893. https://doi.org/10.1016/j.eng.2018.09.007
Zhou, Yuhong, Ziaoping Zhang, and Richard H. Ebright.
1993. Identification of the activating region of catabolite gene
activator protein (CAP): Isolation and characterization of mutants
of CAP specifically defective in transcription activation. Proceedings
of the National Academy of Sciences of the United States of
America 90:6081-6085.
Back to Top